4.2 Iron transport
It is obvious that iron must be transported around the human body. Firstly, it must be transported from the food in the gut to the places where it is required. Mostly, iron is required in the bone marrow, where red blood cells are formed. Red blood cells have a finite lifetime of about only four months, and old cells are destroyed, usually in the spleen. Iron from the destruction of these cells is then transported from the spleen back to the bone marrow to be recycled.
Iron cannot be transported around the body's circulation system as free iron, since it would be susceptible to chelation by siderophores, or may precipitate as iron(III) oxide, or may form iron(II). Therefore, a specific transport protein is required, called transferrin. (In fact, a whole class of transferrin-like proteins is involved in iron transport.) Transferrin is a medium-sized protein with a relative molecular mass of about 80 000. The crystal structures of transferrin with and without iron have been obtained, and the overall structure is shown in Figure 8, The structure without any iron (Figure 8a) shows that transferrin can be considered as two very similar polypeptides back-to-back, with each of the polypeptides having a large cleft. The apex of each cleft coordinates one iron atom; each transferrin molecule is therefore capable of transporting two iron atoms. Also, on the binding of iron, there is a significant change in the higher-order structure of the protein, such that the two sides of the cleft corne together and incarcerate the iron atoms (Figure 8b). Both iron atoms are now buried deep within the protein structure. (It is not fully clear why the iron atoms are buried in this way, but it may help in protecting the iron atom from microbial siderophores.)
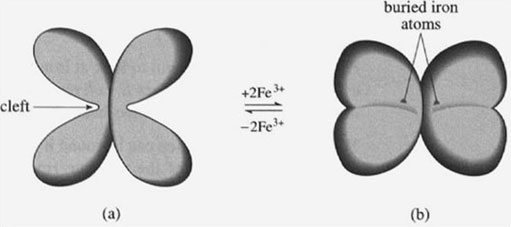
The iron binding site is rich in hard ligands, which are suitable for binding iron(III) in a stable complex (Figure 9a). When the iron atom enters the active site (Figure 9b) it is coordinated by one η1-aspartyl, one histidyl and two tyrosinate side-chains; a non-protein ligand also coordinates to it. This external ligand is a carbonate, CO32−, which is held in place within the protein via hydrogen bonds to the protein backbone.
Activity 15
What is the mode of bonding of the CO32− group?
Answer
The carbonate coordinates to the iron in an η2 fashion; in other words, it is a chelating ligand.
Once the carbonate is held in place, we can see another example of ligand preorganisation, where an octahedral environment of hard/borderline Iigands is ready to receive the iron(III) ion. The carbonate binding appears to facilitate the iron binding by the protein and vice versa, and so the system is said to be synergistic. It is not clear why this unusual synergistic binding of iron and carbonate occurs in transferrin, but it may have something to do with the way iron is released from transferrin.
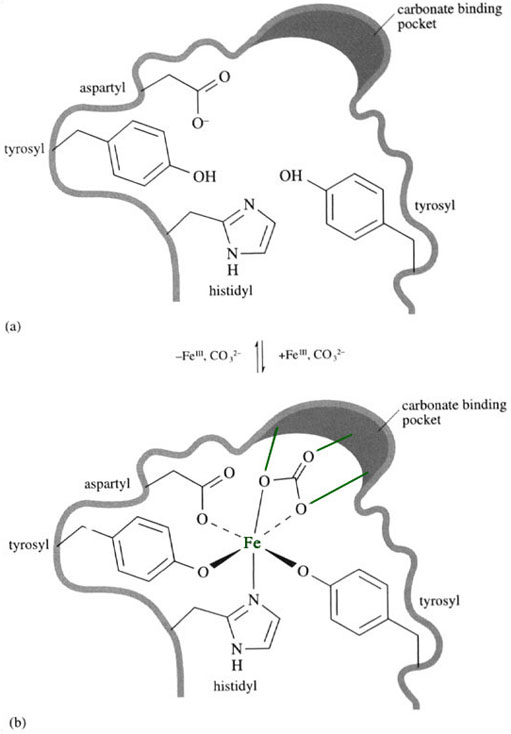
Since all the coordinating ligands in transferrin can be considered as hard or ‘borderline’ ligands, it is no surprise that transferrin forms a very stable complex with iron(III). The stability constant of the Fe(III)–transferrin complex is c. 1020 mol−1 l. This is high enough to protect the iron(III) against the low concentration of any siderophores present. Indeed, the transferrins show mild antibacterial properties, in which their method of operation is to prevent extensive iron chelation by siderophores (see Box 2).
Box 2 Iron in human milk
It has been known for some time that bottle-fed babies are more likely to suffer from gastric infections than breast-fed babies; this may be despite strict hygiene standards. The reason for this probably lies in the availability of iron within the baby's feed. Breast milk is known to contain a transferrin-like protein called lactoferrin. The lactoferrin chelates all the iron in the mother's milk, and prevents iron chelation by microbial siderophores. Formula milk, on the other hand, does not contain human lactoferrin, so the iron in the feed is more available for chelation by siderophores secreted by bacteria.
Transferrin also forms relatively stable complexes with other hard metals (Table 2). Current thinking suggests that these other metals are transported by transferrin into cells, where they are potentially detrimental. One element in particular, aluminium, is of concern because it is used widely in cooking utensils.
Metal | Log10(stability constant) |
---|---|
cadmium(II) | 5.95 |
zinc(II) | 7.80 |
aluminium(III) | 13.50 |
iron(III) | 22.80 |
Therefore, we can see from the structure and function of transferrin that the transport of iron within (and without) the body is very carefully managed, so as not to allow any free soluble iron to form. How, then, is iron stored? After all, we must store iron, since we need a reservoir of it for the synthesis of iron-containing proteins, most notably haemoglobin and myoglobin. As with iron transport, the iron storage systems need to ensure that free, soluble iron is not formed.